Photovoltaics



Photovoltaics (PV) is the conversion of light into electricity using semiconducting materials that exhibit the photovoltaic effect, a phenomenon studied in physics, photochemistry, and electrochemistry. The photovoltaic effect is commercially used for electricity generation and as photosensors.
A photovoltaic system employs solar modules, each comprising a number of solar cells, which generate electrical power. PV installations may be ground-mounted, rooftop-mounted, wall-mounted or floating. The mount may be fixed or use a solar tracker to follow the sun across the sky.
Photovoltaic technology helps to mitigate climate change because it emits much less carbon dioxide than fossil fuels. Solar PV has specific advantages as an energy source: once installed, its operation does not generate any pollution or any greenhouse gas emissions; it shows scalability in respect of power needs and silicon has large availability in the Earth's crust, although other materials required in PV system manufacture such as silver may constrain further growth in the technology. Other major constraints identified include competition for land use.[1] The use of PV as a main source requires energy storage systems or global distribution by high-voltage direct current power lines causing additional costs, and also has a number of other specific disadvantages such as variable power generation which have to be balanced. Production and installation does cause some pollution and greenhouse gas emissions, though only a fraction of the emissions caused by fossil fuels.[2]
Photovoltaic systems have long been used in specialized applications as stand-alone installations and grid-connected PV systems have been in use since the 1990s.[3] Photovoltaic modules were first mass-produced in 2000, when the German government funded a one hundred thousand roof program.[4] Decreasing costs has allowed PV to grow as an energy source. This has been partially driven by massive Chinese government investment in developing solar production capacity since 2000, and achieving economies of scale. Improvements in manufacturing technology and efficiency have also led to decreasing costs.[5][6] Net metering and financial incentives, such as preferential feed-in tariffs for solar-generated electricity, have supported solar PV installations in many countries.[7] Panel prices dropped by a factor of 4 between 2004 and 2011. Module prices dropped by about 90% over the 2010s.
In 2022, worldwide installed PV capacity increased to more than 1 terawatt (TW) covering nearly two percent of global electricity demand.[8] After hydro and wind powers, PV is the third renewable energy source in terms of global capacity. In 2022, the International Energy Agency expected a growth by over 1 TW from 2022 to 2027.[9] In some instances, PV has offered the cheapest source of electrical power in regions with a high solar potential, with a bid for pricing as low as 0.015 US$/kWh in Qatar in 2023.[10] In 2023, the International Energy Agency stated in its World Energy Outlook that '[f]or projects with low cost financing that tap high quality resources, solar PV is now the cheapest source of electricity in history.[11]
Etymology
[edit]The term "photovoltaic" comes from the Greek φῶς (phōs) meaning "light", and from "volt", the unit of electromotive force, the volt, which in turn comes from the last name of the Italian physicist Alessandro Volta, inventor of the battery (electrochemical cell). The term "photovoltaic" has been in use in English since 1849.[12]
History
[edit]In 1989, the German Research Ministry initiated the first ever program to finance PV roofs (2200 roofs). A program led by Walter Sandtner in Bonn, Germany.[13]
In 1994, Japan followed in their footsteps and conducted a similar program with 539 residential PV systems installed.[14] Since, many countries have continued to produce and finance PV systems in an exponential speed.
Solar cells
[edit]

Photovoltaics are best known as a method for generating electric power by using solar cells to convert energy from the sun into a flow of electrons by the photovoltaic effect.[15][16]
Solar cells produce direct current electricity from sunlight which can be used to power equipment or to recharge batteries. The first practical application of photovoltaics was to power orbiting satellites and other spacecraft, but today the majority of photovoltaic modules are used for grid-connected systems for power generation. In this case an inverter is required to convert the DC to AC. There is also a smaller market for stand alone systems for remote dwellings, boats, recreational vehicles, electric cars, roadside emergency telephones, remote sensing, and cathodic protection of pipelines.
Photovoltaic power generation employs solar modules composed of a number of solar cells containing a semiconductor material.[17] Copper solar cables connect modules (module cable), arrays (array cable), and sub-fields. Because of the growing demand for renewable energy sources, the manufacturing of solar cells and photovoltaic arrays has advanced considerably in recent years.[18][19][20]
Cells require protection from the environment and are usually packaged tightly in solar modules.
Photovoltaic module power is measured under standard test conditions (STC) in "Wp" (watts peak).[21] The actual power output at a particular place may be less than or greater than this rated value, depending on geographical location, time of day, weather conditions, and other factors.[22] Solar photovoltaic array capacity factors are typically under 25% when not coupled with storage, which is lower than many other industrial sources of electricity.[23]
Solar cell efficiencies
[edit]
Solar-cell efficiency is the portion of energy in the form of sunlight that can be converted via photovoltaics into electricity by the solar cell.
The efficiency of the solar cells used in a photovoltaic system, in combination with latitude and climate, determines the annual energy output of the system. For example, a solar panel with 20% efficiency and an area of 1 m2 produces 200 kWh/yr at Standard Test Conditions if exposed to the Standard Test Condition solar irradiance value of 1000 W/m2 for 2.74 hours a day. Usually solar panels are exposed to sunlight for longer than this in a given day, but the solar irradiance is less than 1000 W/m2 for most of the day. A solar panel can produce more when the Sun is high in Earth's sky and produces less in cloudy conditions, or when the Sun is low in the sky. The Sun is lower in the sky in the winter.
Two location dependent factors that affect solar PV yield are the dispersion and intensity of solar radiation. These two variables can vary greatly between each country.[24] The global regions that have high radiation levels throughout the year are the middle east, Northern Chile, Australia, China, and Southwestern USA.[24][25] In a high-yield solar area like central Colorado, which receives annual insolation of 2000 kWh/m2/year,[26] a panel can be expected to produce 400 kWh of energy per year. However, in Michigan, which receives only 1400 kWh/m2/year,[26] annual energy yield drops to 280 kWh for the same panel. At more northerly European latitudes, yields are significantly lower: 175 kWh annual energy yield in southern England under the same conditions.[27]
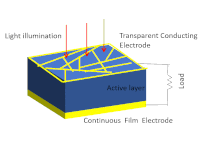
Several factors affect a cell's conversion efficiency, including its reflectance, thermodynamic efficiency, charge carrier separation efficiency, charge carrier collection efficiency and conduction efficiency values.[29][28] Because these parameters can be difficult to measure directly, other parameters are measured instead, including quantum efficiency, open-circuit voltage (VOC) ratio, and § Fill factor. Reflectance losses are accounted for by the quantum efficiency value, as they affect external quantum efficiency. Recombination losses are accounted for by the quantum efficiency, VOC ratio, and fill factor values. Resistive losses are predominantly accounted for by the fill factor value, but also contribute to the quantum efficiency and VOC ratio values.
As of 2024, the world record for solar cell efficiency is 47.6%, set in May 2022 by Fraunhofer ISE, with a III-V four-junction concentrating photovoltaic (CPV) cell.[30][31] This beat the previous record of 47.1%, set in 2019 by multi-junction concentrator solar cells developed at National Renewable Energy Laboratory (NREL), Golden, Colorado, USA,[32] which was set in lab conditions, under extremely concentrated light. The record in real-world conditions is held by NREL, who developed triple junction cells with a tested efficiency of 39.5%.[33][34]Performance and degradation
[edit]
Module performance is generally rated under standard test conditions (STC): irradiance of 1,000 W/m2, solar spectrum of AM 1.5 and module temperature at 25 °C.[35] The actual voltage and current output of the module changes as lighting, temperature and load conditions change, so there is never one specific voltage at which the module operates. Performance varies depending on geographic location, time of day, the day of the year, amount of solar irradiance, direction and tilt of modules, cloud cover, shading, soiling, state of charge, and temperature. Performance of a module or panel can be measured at different time intervals with a DC clamp meter or shunt and logged, graphed, or charted with a chart recorder or data logger.
For optimum performance, a solar panel needs to be made of similar modules oriented in the same direction perpendicular to direct sunlight. Bypass diodes are used to circumvent broken or shaded panels and optimize output. These bypass diodes are usually placed along groups of solar cells to create a continuous flow.[36]
Electrical characteristics include nominal power (PMAX, measured in W), open-circuit voltage (VOC), short-circuit current (ISC, measured in amperes), maximum power voltage (VMPP), maximum power current (IMPP), peak power (watt-peak, Wp), and module efficiency (%).
Open-circuit voltage or VOC is the maximum voltage the module can produce when not connected to an electrical circuit or system.[37] VOC can be measured with a voltmeter directly on an illuminated module's terminals or on its disconnected cable.
The peak power rating, Wp, is the maximum output under standard test conditions (not the maximum possible output). Typical modules, which could measure approximately 1 by 2 metres (3 ft × 7 ft), will be rated from as low as 75 W to as high as 600 W, depending on their efficiency. At the time of testing, the test modules are binned according to their test results, and a typical manufacturer might rate their modules in 5 W increments, and either rate them at +/- 3%, +/-5%, +3/-0% or +5/-0%.[38][39][40]
Influence of temperature
[edit]The performance of a photovoltaic (PV) module depends on the environmental conditions, mainly on the global incident irradiance G in the plane of the module. However, the temperature T of the p–n junction also influences the main electrical parameters: the short circuit current ISC, the open circuit voltage VOC and the maximum power Pmax. In general, it is known that VOC shows a significant inverse correlation with T, while for ISC this correlation is direct, but weaker, so that this increase does not compensate for the decrease in VOC. As a consequence, Pmax decreases when T increases. This correlation between the power output of a solar cell and the working temperature of its junction depends on the semiconductor material, and is due to the influence of T on the concentration, lifetime, and mobility of the intrinsic carriers, i.e., electrons and gaps. inside the photovoltaic cell.
Temperature sensitivity is usually described by temperature coefficients, each of which expresses the derivative of the parameter to which it refers with respect to the junction temperature. The values of these parameters, which can be found in any data sheet of the photovoltaic module, are the following:
- β: VOC variation coefficient with respect to T, given by ∂VOC/∂T.
- α: Coefficient of variation of ISC with respect to T, given by ∂ISC/∂T.
- δ: Coefficient of variation of Pmax with respect to T, given by ∂Pmax/∂T.
Techniques for estimating these coefficients from experimental data can be found in the literature.[41]
Degradation
[edit]The ability of solar modules to withstand damage by rain, hail, heavy snow load, and cycles of heat and cold varies by manufacturer, although most solar panels on the U.S. market are UL listed, meaning they have gone through testing to withstand hail.[42]
Potential-induced degradation (also called PID) is a potential-induced performance degradation in crystalline photovoltaic modules, caused by so-called stray currents.[43] This effect may cause power loss of up to 30%.[44]
The largest challenge for photovoltaic technology is the purchase price per watt of electricity produced. Advancements in photovoltaic technologies have brought about the process of "doping" the silicon substrate to lower the activation energy thereby making the panel more efficient in converting photons to retrievable electrons.[45]
Chemicals such as boron (p-type) are applied into the semiconductor crystal in order to create donor and acceptor energy levels substantially closer to the valence and conductor bands.[46] In doing so, the addition of boron impurity allows the activation energy to decrease twenty-fold from 1.12 eV to 0.05 eV. Since the potential difference (EB) is so low, the boron is able to thermally ionize at room temperatures. This allows for free energy carriers in the conduction and valence bands thereby allowing greater conversion of photons to electrons.
The power output of a photovoltaic (PV) device decreases over time. This decrease is due to its exposure to solar radiation as well as other external conditions. The degradation index, which is defined as the annual percentage of output power loss, is a key factor in determining the long-term production of a photovoltaic plant. To estimate this degradation, the percentage of decrease associated with each of the electrical parameters. The individual degradation of a photovoltaic module can significantly influence the performance of a complete string. Furthermore, not all modules in the same installation decrease their performance at exactly the same rate. Given a set of modules exposed to long-term outdoor conditions, the individual degradation of the main electrical parameters and the increase in their dispersion must be considered. As each module tends to degrade differently, the behavior of the modules will be increasingly different over time, negatively affecting the overall performance of the plant.
There are several studies dealing with the power degradation analysis of modules based on different photovoltaic technologies available in the literature. According to a recent study,[47] the degradation of crystalline silicon modules is very regular, oscillating between 0.8% and 1.0% per year.
On the other hand, if we analyze the performance of thin-film photovoltaic modules, an initial period of strong degradation is observed (which can last several months and up to two years), followed by a later stage in which the degradation stabilizes, being then comparable to that of crystalline silicon.[48] Strong seasonal variations are also observed in such thin-film technologies because the influence of the solar spectrum is much greater. For example, for modules of amorphous silicon, micromorphic silicon or cadmium telluride, we are talking about annual degradation rates for the first years of between 3% and 4%.[49] However, other technologies, such as CIGS, show much lower degradation rates, even in those early years.
Manufacturing of PV systems
[edit]Overall the manufacturing process of creating solar photovoltaics is simple in that it does not require the culmination of many complex or moving parts. Because of the solid-state nature of PV systems, they often have relatively long lifetimes, anywhere from 10 to 30 years. To increase the electrical output of a PV system, the manufacturer must simply add more photovoltaic components. Because of this, economies of scale are important for manufacturers as costs decrease with increasing output.[50]
While there are many types of PV systems known to be effective, crystalline silicon PV accounted for around 90% of the worldwide production of PV in 2013. Manufacturing silicon PV systems has several steps. First, polysilicon is processed from mined quartz until it is very pure (semi-conductor grade). This is melted down when small amounts of boron, a group III element, are added to make a p-type semiconductor rich in electron holes. Typically using a seed crystal, an ingot of this solution is grown from the liquid polycrystalline. The ingot may also be cast in a mold. Wafers of this semiconductor material are cut from the bulk material with wire saws, and then go through surface etching before being cleaned. Next, the wafers are placed into a phosphorus vapor deposition furnace which lays a very thin layer of phosphorus, a group V element, which creates an n-type semiconducting surface. To reduce energy losses, an anti-reflective coating is added to the surface, along with electrical contacts. After finishing the cell, cells are connected via electrical circuit according to the specific application and prepared for shipping and installation.[51]
Environmental costs of manufacture
[edit]Solar photovoltaic power is not entirely "clean energy": production produces greenhouse gas emissions, materials used to build the cells are potentially unsustainable and will run out eventually,[clarification needed][citation needed] the technology uses toxic substances which cause pollution,[citation needed] and there are no viable technologies for recycling solar waste.[52][obsolete source] Data required to investigate their impact are sometimes affected by a rather large amount of uncertainty. The values of human labor and water consumption, for example, are not precisely assessed due to the lack of systematic and accurate analyses in the scientific literature.[1] One difficulty in determining effects due to PV is to determine if the wastes are released to the air, water, or soil during the manufacturing phase.[53][obsolete source] Life-cycle assessments, which look at all different environment effects ranging from global warming potential, pollution, water depletion and others, are unavailable for PV. Instead, studies have tried to estimate the impact and potential impact of various types of PV, but these estimates are usually restricted to simply assessing energy costs of the manufacture and/or transport, because these are new technologies and the total environmental impact of their components and disposal methods are unknown, even for commercially available first generation solar cells, let alone experimental prototypes with no commercial viability.[54][better source needed]
Thus, estimates of the environmental impact of PV have focused on carbon dioxide equivalents per kWh or energy pay-back time (EPBT).[citation needed] The EPBT describes the timespan a PV system needs to operate in order to generate the same amount of energy that was used for its manufacture.[55] Another study includes transport energy costs in the EPBT.[56] The EPBT has also been defined completely differently as "the time needed to compensate for the total renewable- and non-renewable primary energy required during the life cycle of a PV system" in another study, which also included installation costs.[57] This energy amortization, given in years, is also referred to as break-even energy payback time.[58] The lower the EPBT, the lower the environmental cost of solar power.[citation needed] The EPBT depends vastly on the location where the PV system is installed (e.g. the amount of sunlight available and the efficiency of the electrical grid)[56] and on the type of system, namely the system's components.[55]
A 2015 review of EPBT estimates of first and second-generation PV suggested that there was greater variation in embedded energy than in efficiency of the cells implying that it was mainly the embedded energy that needs to reduce to have a greater reduction in EPBT.[59]
In general, the most important component of solar panels, which accounts for much of the energy use and greenhouse gas emissions, is the refining of the polysilicon.[55] As to how much percentage of the EPBT this silicon depends on the type of system. A fully autarkic system requires additional components ('Balance of System', the power inverters, storage, etc.) which significantly increase the energy cost of manufacture, but in a simple rooftop system, some 90% of the energy cost is from silicon, with the remainder coming from the inverters and module frame.[55]
The EPBT relates closely to the concepts of net energy gain (NEG) and energy returned on energy invested (EROI). They are both used in energy economics and refer to the difference between the energy expended to harvest an energy source and the amount of energy gained from that harvest. The NEG and EROI also take the operating lifetime of a PV system into account and a working life of 25 to 30 years is typically assumed. From these metrics, the Energy payback Time can be derived by calculation.[60][61]
EPBT improvements
[edit]PV systems using crystalline silicon, by far the majority of the systems in practical use, have such a high EPBT because silicon is produced by the reduction of high-grade quartz sand in electric furnaces. This coke-fired smelting process occurs at high temperatures of more than 1000 °C and is very energy intensive, using about 11 kilowatt-hours (kWh) per produced kilogram of silicon.[62] The energy requirements of this process makes the energy cost per unit of silicon produced relatively inelastic, which means that the production process itself will not become more efficient in the future.
Nonetheless, the energy payback time has shortened significantly over the last years, as crystalline silicon cells became ever more efficient in converting sunlight, while the thickness of the wafer material was constantly reduced and therefore required less silicon for its manufacture. Within the last ten years, the amount of silicon used for solar cells declined from 16 to 6 grams per watt-peak. In the same period, the thickness of a c-Si wafer was reduced from 300 μm, or microns, to about 160–190 μm. The sawing techniques that slice crystalline silicon ingots into wafers have also improved by reducing the kerf loss and making it easier to recycle the silicon sawdust.[63][64]
Parameter | Mono-Si | CdTe |
---|---|---|
Cell efficiency | 16.5% | 15.6% |
Derate cell to module efficiency | 8.5% | 13.9% |
Module efficiency | 15.1% | 13.4% |
Wafer thickness / layer thickness | 190 μm | 4.0 μm |
Kerf loss | 190 μm | – |
Silver per cell | 9.6 g/m2 | – |
Glass thickness | 4.0 mm | 3.5 mm |
Operational lifetime | 30 years | 30 years |
Source: IEA-PVPS, Life Cycle Assessment, March 2015[65] |
Effects from first generation PV
[edit]Crystalline silicon modules are the most extensively studied PV type in terms of LCA since they are the most commonly used. Mono-crystalline silicon photovoltaic systems (mono-si) have an average efficiency of 14.0%.[66] The cells tend to follow a structure of front electrode, anti-reflection film, n-layer, p-layer, and back electrode, with the sun hitting the front electrode. EPBT ranges from 1.7 to 2.7 years.[67] The cradle to gate of CO2-eq/kWh ranges from 37.3 to 72.2 grams when installed in Southern Europe.[68]
Techniques to produce multi-crystalline silicon (multi-si) photovoltaic cells are simpler and cheaper than mono-si, however tend to make less efficient cells, an average of 13.2%.[66] EPBT ranges from 1.5 to 2.6 years.[67] The cradle to gate of CO2-eq/kWh ranges from 28.5 to 69 grams when installed in Southern Europe.[68]
Assuming that the following countries had a high-quality grid infrastructure as in Europe, in 2020 it was calculated it would take 1.28 years in Ottawa, Canada, for a rooftop photovoltaic system to produce the same amount of energy as required to manufacture the silicon in the modules in it (excluding the silver, glass, mounts and other components), 0.97 years in Catania, Italy, and 0.4 years in Jaipur, India. Outside of Europe, where net grid efficiencies are lower, it would take longer. This 'energy payback time' can be seen as the portion of time during the useful lifetime of the module in which the energy production is polluting. At best, this means that a 30-year old panel has produced clean energy for 97% of its lifetime, or that the silicon in the modules in a solar panel produce 97% less greenhouse gas emissions than a coal-fired plant for the same amount of energy (assuming and ignoring many things).[56] Some studies have looked beyond EPBT and GWP to other environmental effects. In one such study, conventional energy mix in Greece was compared to multi-si PV and found a 95% overall reduction in effects including carcinogens, eco-toxicity, acidification, eutrophication, and eleven others.[69]
Impact from second generation PV
[edit]Cadmium telluride (CdTe) is one of the fastest-growing thin film based solar cells which are collectively known as second-generation devices. This new thin-film device also shares similar performance restrictions (Shockley-Queisser efficiency limit) as conventional Si devices but promises to lower the cost of each device by both reducing material and energy consumption during manufacturing. The global market share of CdTe was 4.7% in 2008.[53] This technology's highest power conversion efficiency is 21%.[70] The cell structure includes glass substrate (around 2 mm), transparent conductor layer, CdS buffer layer (50–150 nm), CdTe absorber and a metal contact layer.
CdTe PV systems require less energy input in their production than other commercial PV systems per unit electricity production. The average CO2-eq/kWh is around 18 grams (cradle to gate). CdTe has the fastest EPBT of all commercial PV technologies, which varies between 0.3 and 1.2 years.[71]
Effects from third generation PV
[edit]Third-generation PVs are designed to combine the advantages of both the first and second generation devices and they do not have Shockley-Queisser limit, a theoretical limit for first and second generation PV cells. The thickness of a third generation device is less than 1 μm.[72]
Two new promising thin film technologies are copper zinc tin sulfide (Cu2ZnSnS4 or CZTS),[54] zinc phosphide (Zn3P2)[54] and single-walled carbon nano-tubes (SWCNT).[73] These thin films are currently only produced in the lab but may be commercialized in the future. The manufacturing of CZTS and (Zn3P2) processes are expected to be similar to those of current thin film technologies of CIGS and CdTe, respectively. While the absorber layer of SWCNT PV is expected to be synthesized with CoMoCAT method.[74] by Contrary to established thin films such as CIGS and CdTe, CZTS, Zn3P2, and SWCNT PVs are made from earth abundant, nontoxic materials and have the potential to produce more electricity annually than the current worldwide consumption.[75][76] While CZTS and Zn3P2 offer good promise for these reasons, the specific environmental implications of their commercial production are not yet known. Global warming potential of CZTS and Zn3P2 were found 38 and 30 grams CO2-eq/kWh while their corresponding EPBT were found 1.85 and 0.78 years, respectively.[54] Overall, CdTe and Zn3P2 have similar environmental effects but can slightly outperform CIGS and CZTS.[54] A study on environmental impacts of SWCNT PVs by Celik et al., including an existing 1% efficient device and a theoretical 28% efficient device, found that, compared to monocrystalline Si, the environmental impacts from 1% SWCNT was ~18 times higher due mainly to the short lifetime of three years.[73]
Economics
[edit]![]() Source: Apricus[77] |
There have been major changes in the underlying costs, industry structure and market prices of solar photovoltaics technology, over the years, and gaining a coherent picture of the shifts occurring across the industry value chain globally is a challenge. This is due to: "the rapidity of cost and price changes, the complexity of the PV supply chain, which involves a large number of manufacturing processes, the balance of system (BOS) and installation costs associated with complete PV systems, the choice of different distribution channels, and differences between regional markets within which PV is being deployed". Further complexities result from the many different policy support initiatives that have been put in place to facilitate photovoltaics commercialisation in various countries.[3]
Renewable energy technologies have generally gotten cheaper since their invention.[78][79][80] Renewable energy systems have become cheaper to build than fossil fuel power plants across much of the world, thanks to advances in wind and solar energy technology, in particular.[81]
- Implications for electricity bill management and energy investment
There is no silver bullet in electricity or energy demand and bill management, because customers (sites) have different specific situations, e.g. different comfort/convenience needs, different electricity tariffs, or different usage patterns. Electricity tariff may have a few elements, such as daily access and metering charge, energy charge (based on kWh, MWh) or peak demand charge (e.g. a price for the highest 30min energy consumption in a month). PV is a promising option for reducing energy charges when electricity prices are reasonably high and continuously increasing, such as in Australia and Germany. However, for sites with peak demand charge in place, PV may be less attractive if peak demands mostly occur in the late afternoon to early evening, for example in residential communities. Overall, energy investment is largely an economic decision and it is better to make investment decisions based on systematic evaluation of options in operational improvement, energy efficiency, onsite generation and energy storage.[82][83]
Hardware costs
[edit]![]() | This section may contain an excessive amount of intricate detail that may interest only a particular audience. (December 2024) |
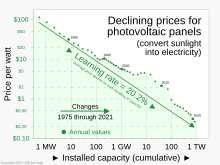
In 1977 crystalline silicon solar cell prices were at $76.67/W.[85]
Although wholesale module prices remained flat at around $3.50 to $4.00/W in the early 2000s due to high demand in Germany and Spain afforded by generous subsidies and shortage of polysilicon, demand crashed with the abrupt ending of Spanish subsidies after the market crash of 2008, and the price dropped rapidly to $2.00/W. Manufacturers were able to maintain a positive operating margin despite a 50% drop in income due to innovation and reductions in costs. In late 2011, factory-gate prices for crystalline-silicon photovoltaic modules suddenly dropped below the $1.00/W mark, taking many in the industry by surprise, and has caused a number of solar manufacturing companies to go bankrupt throughout the world. The $1.00/W cost is often regarded in the PV industry as marking the achievement of grid parity for PV, but most experts do not believe this price point is sustainable. Technological advancements, manufacturing process improvements, and industry re-structuring, may mean that further price reductions are possible.[3] The average retail price of solar cells as monitored by the Solarbuzz group fell from $3.50/watt to $2.43/watt over the course of 2011.[86] In 2013 wholesale prices had fallen to $0.74/W.[85] This has been cited as evidence supporting 'Swanson's law', an observation similar to the famous Moore's Law, which claims that solar cell prices fall 20% for every doubling of industry capacity.[85] The Fraunhofer Institute defines the 'learning rate' as the drop in prices as the cumulative production doubles, some 25% between 1980 and 2010. Although the prices for modules have dropped quickly, current inverter prices have dropped at a much lower rate, and in 2019 constitute over 61% of the cost per kWp, from a quarter in the early 2000s.[56]
Note that the prices mentioned above are for bare modules, another way of looking at module prices is to include installation costs. In the US, according to the Solar Energy Industries Association, the price of installed rooftop PV modules for homeowners fell from $9.00/W in 2006 to $5.46/W in 2011. Including the prices paid by industrial installations, the national installed price drops to $3.45/W. This is markedly higher than elsewhere in the world, in Germany homeowner rooftop installations averaged at $2.24/W. The cost differences are thought to be primarily based on the higher regulatory burden and lack of a national solar policy in the US.[87]
By the end of 2012 Chinese manufacturers had production costs of $0.50/W in the cheapest modules.[88] In some markets distributors of these modules can earn a considerable margin, buying at factory-gate price and selling at the highest price the market can support ('value-based pricing').[3] In California PV reached grid parity in 2011, which is usually defined as PV production costs at or below retail electricity prices (though often still above the power station prices for coal or gas-fired generation without their distribution and other costs).[89] Grid parity had been reached in 19 markets in 2014.[90][91]
By 2024, massive increases of production of solar panels in China had caused module prices to drop to as low as $0.11/W, an over 90 percent reduction from 2011 prices.[92]
Levelised cost of electricity
[edit]
The levelised cost of electricity (LCOE) is the cost per kWh based on the costs distributed over the project lifetime, and is thought to be a better metric for calculating viability than price per wattage. LCOEs vary dramatically depending on the location.[3] The LCOE can be considered the minimum price customers will have to pay the utility company in order for it to break even on the investment in a new power station.[5] Grid parity is roughly achieved when the LCOE falls to a similar price as conventional local grid prices, although in actuality the calculations are not directly comparable.[93] Large industrial PV installations had reached grid parity in California in 2011.[80][93] Grid parity for rooftop systems was still believed to be much farther away at this time.[93] Many LCOE calculations are not thought to be accurate, and a large amount of assumptions are required.[3][93] Module prices may drop further, and the LCOE for solar may correspondingly drop in the future.[94]
Because energy demands rise and fall over the course of the day, and solar power is limited by the fact that the sun sets, solar power companies must also factor in the additional costs of supplying a more stable alternative energy supplies to the grid in order to stabilize the system, or storing the energy. These costs are not factored into LCOE calculations, nor are special subsidies or premiums that may make buying solar power more attractive.[5] The unreliability and temporal variation in generation of solar and wind power is a major problem. Too much of these volatile power sources can cause instability of the entire grid.[95]
As of 2017 power-purchase agreement prices for solar farms below $0.05/kWh are common in the United States, and the lowest bids in some Persian Gulf countries were about $0.03/kWh.[96] The goal of the United States Department of Energy is to achieve a levelised cost of energy for solar PV of $0.03/kWh for utility companies.[97]
Subsidies and financing
[edit]Financial incentives for photovoltaics, such as feed-in tariffs (FITs), were often offered to electricity consumers to install and operate solar-electric generating systems, and in some countries such subsidies are the only way photovoltaics can remain economically profitable.[98][obsolete source] PV FITs were crucial for early growth of photovoltaics. Germany and Spain were the most important countries offering subsidies for PV, and the policies of these countries drove demand.[3]
Some US solar cell manufacturing companies have repeatedly complained that the dropping prices of PV module costs have been achieved due to subsidies by the government of China, and the dumping of these products below fair market prices. US manufacturers generally recommend high tariffs on foreign supplies to allow them remain profitable. In response to these concerns, the Obama administration began to levy tariffs on US consumers of these products in 2012 to raise prices for domestic manufacturers.[3] The USA, however, also subsidies the industry.[87]
Some environmentalists have promoted the idea that government incentives should be used in order to expand the PV manufacturing industry to reduce costs of PV-generated electricity much more rapidly to a level where it is able to compete with fossil fuels in a free market.[citation needed] This is based on the theory that when the manufacturing capacity doubles, economies of scale will cause the prices of the solar products to halve.[5]
In many countries access to capital is lacking to develop PV projects.[99] To solve this problem, securitization is sometimes used to accelerate development of solar photovoltaic projects.[89][100][101]
Other
[edit]Photovoltaic power is also generated during a time of day that is close to peak demand (precedes it) in electricity systems with high use of air conditioning. Since large-scale PV operation requires back-up in the form of spinning reserves, its[clarification needed] marginal cost of generation in the middle of the day is typically lowest, but not zero, when PV is generating electricity. This can be seen in Figure 1 of this paper:.[102] For residential properties with private PV facilities networked to the grid, the owner may be able earn extra money when the time of generation is included, as electricity is worth more during the day than at night.[103]
One journalist theorised in 2012 that if the energy bills of Americans were forced upwards by imposing an extra tax of $50/ton on carbon dioxide emissions from coal-fired power, this could have allowed solar PV to appear more cost-competitive to consumers in most locations.[86]
Growth
[edit]
Solar photovoltaics formed the largest body of research among the seven sustainable energy types examined in a global bibliometric study, with the annual scientific output growing from 9,094 publications in 2011 to 14,447 publications in 2019.[104]
Likewise, the application of solar photovoltaics is growing rapidly and the worldwide installed capacity reached one terawatt in April 2022.[105] The total power output of the world's PV capacity in a calendar year is now beyond 500 TWh of electricity. This represents 2% of worldwide electricity demand. More than 100 countries, such as Brazil and India, use solar PV.[106][107] China is followed by the United States and Japan, while installations in Germany, once the world's largest producer, have been slowing down.
Honduras generated the highest percentage of its energy from solar in 2019, 14.8%.[108] As of 2019, Vietnam has the highest installed capacity in Southeast Asia, about 4.5 GW.[109] The annualized installation rate of about 90 W per capita per annum places Vietnam among world leaders.[109] Generous Feed-in tariff (FIT) and government supporting policies such as tax exemptions were the key to enable Vietnam's solar PV boom. Underlying drivers include the government's desire to enhance energy self-sufficiency and the public's demand for local environmental quality.[109]
A key barrier is limited transmission grid capacity.[109]
China has the world's largest solar power capacity, with 390 GW of installed capacity in 2022 compared with about 200 GW in the European Union, according to International Energy Agency data.[110] Other countries with the world's largest solar power capacities include the United States, Japan and Germany.
| ||||||||||||||||||||||||||||||||||||||||||||||||||||||||||||||||||||||||||||||||||||
Data: IEA-PVPS Snapshot of Global PV Markets 2023 report, April 2023[111] |
In 2017, it was thought probable that by 2030 global PV installed capacities could be between 3,000 and 10,000 GW.[96] Greenpeace in 2010 claimed that 1,845 GW of PV systems worldwide could be generating approximately 2,646 TWh/year of electricity by 2030, and by 2050 over 20% of all electricity could be provided by PV.[112]
Applications
[edit]There are many practical applications for the use of solar panels or photovoltaics covering every technological domain under the sun. From the fields of the agricultural industry as a power source for irrigation to its usage in remote health care facilities to refrigerate medical supplies. Other applications include power generation at various scales and attempts to integrate them into homes and public infrastructure. PV modules are used in photovoltaic systems and include a large variety of electrical devices.
Photovoltaic systems
[edit]A photovoltaic system, or solar PV system is a power system designed to supply usable solar power by means of photovoltaics. It consists of an arrangement of several components, including solar panels to absorb and directly convert sunlight into electricity, a solar inverter to change the electric current from DC to AC, as well as mounting, cabling and other electrical accessories. PV systems range from small, roof-top mounted or building-integrated systems with capacities from a few to several tens of kilowatts, to large utility-scale power stations of hundreds of megawatts. Nowadays, most PV systems are grid-connected, while stand-alone systems only account for a small portion of the market.
Photo sensors
[edit]Photosensors are sensors of light or other electromagnetic radiation.[113] A photo detector has a p–n junction that converts light photons into current. The absorbed photons make electron–hole pairs in the depletion region. Photodiodes and photo transistors are a few examples of photo detectors. Solar cells convert some of the light energy absorbed into electrical energy.
Experimental technology
[edit]Crystalline silicon photovoltaics are only one type of PV, and while they represent the majority of solar cells produced currently there are many new and promising technologies that have the potential to be scaled up to meet future energy needs. As of 2018, crystalline silicon cell technology serves as the basis for several PV module types, including monocrystalline, multicrystalline, mono PERC, and bifacial.[114]
Another newer technology, thin-film PV, are manufactured by depositing semiconducting layers of perovskite, a mineral with semiconductor properties, on a substrate in vacuum. The substrate is often glass or stainless-steel, and these semiconducting layers are made of many types of materials including cadmium telluride (CdTe), copper indium diselenide (CIS), copper indium gallium diselenide (CIGS), and amorphous silicon (a-Si). After being deposited onto the substrate the semiconducting layers are separated and connected by electrical circuit by laser scribing.[115][116] Perovskite solar cells are a very efficient solar energy converter and have excellent optoelectronic properties for photovoltaic purposes, but their upscaling from lab-sized cells to large-area modules is still under research.[117] Thin-film photovoltaic materials may possibly become attractive in the future, because of the reduced materials requirements and cost to manufacture modules consisting of thin-films as compared to silicon-based wafers.[118] In 2019 university labs at Oxford, Stanford and elsewhere reported perovskite solar cells with efficiencies of 20-25%.[119]
CIGS
[edit]Copper indium gallium selenide (CIGS) is a thin film solar cell based on the copper indium diselenide (CIS) family of chalcopyrite semiconductors. CIS and CIGS are often used interchangeably within the CIS/CIGS community. The cell structure includes soda lime glass as the substrate, Mo layer as the back contact, CIS/CIGS as the absorber layer, cadmium sulfide (CdS) or Zn (S,OH)x as the buffer layer, and ZnO:Al as the front contact.[120] CIGS is approximately 1/100 the thickness of conventional silicon solar cell technologies. Materials necessary for assembly are readily available, and are less costly per watt of solar cell. CIGS based solar devices resist performance degradation over time and are highly stable in the field.
Reported global warming potential impacts of CIGS ranges 20.5–58.8 grams CO2-eq/kWh of electricity generated for different solar irradiation (1,700 to 2,200 kWh/m2/y) and power conversion efficiency (7.8 – 9.12%).[121] EPBT ranges from 0.2 to 1.4 years,[71] while harmonized value of EPBT was found 1.393 years.[59] Toxicity is an issue within the buffer layer of CIGS modules because it contains cadmium and gallium.[54][122] CIS modules do not contain any heavy metals.
Perovskite solar cells
[edit]
A perovskite solar cell (PSC) is a type of solar cell that includes a perovskite-structured compound, most commonly a hybrid organic–inorganic lead or tin halide-based material as the light-harvesting active layer.[123][124] Perovskite materials, such as methylammonium lead halides and all-inorganic cesium lead halide, are cheap to produce and simple to manufacture.
Solar-cell efficiencies of laboratory-scale devices using these materials have increased from 3.8% in 2009[125] to 25.7% in 2021 in single-junction architectures,[126][127] and, in silicon-based tandem cells, to 29.8%,[126][128] exceeding the maximum efficiency achieved in single-junction silicon solar cells. Perovskite solar cells have therefore been the fastest-advancing solar technology as of 2016[update].[123] With the potential of achieving even higher efficiencies and very low production costs, perovskite solar cells have become commercially attractive. Core problems and research subjects include their short- and long-term stability.[129]Dye-Sensitized Solar Cells
[edit]Dye-sensitized solar cells (DSCs) are a novel thin film solar cell. These solar cells operate under ambient light better than other photovoltaic technologies. They work with light being absorbed in a sensitizing dye between two charge transport materials. Dye surrounds TiO2 nanoparticles which are in a sintered network.[130] TiO2 acts as conduction band in an n-type semiconductor; the scaffold for adorned dye molecules and transports elections during excitation. For TiO2 DSC technology, sample preparation at high temperatures is very effective because higher temperatures produce more suitable textural properties. Another example of DSCs is the copper complex with Cu (II/I) as a redox shuttle with TMBY (4,4',6,6'-tetramethyl-2,2'bipyridine). DSCs show great performance with artificial and indoor light. From a range of 200 lux to 2,000 lux, these cells operate at conditions of a maximum efficiency of 29.7%.[131]
However, there have been issues with DSCs, many of which come from the liquid electrolyte. The solvent is hazardous, and will permeate most plastics. Because it is liquid, it is unstable to temperature variation, leading to freezing in cold temperatures and expansion in warm temperatures causing failure.[132] Another disadvantage is that the solar cell is not ideal for large scale application because of its low efficiency. Some of the benefits for DSC is that it can be used in a variety of light levels (including cloudy conditions), it has a low production cost, and it does not degrade under sunlight, giving it a longer lifetime then other types of thin film solar cells.
OPV
[edit]Other possible future PV technologies include organic, dye-sensitized and quantum-dot photovoltaics.[133] Organic photovoltaics (OPVs) fall into the thin-film category of manufacturing, and typically operate around the 12% efficiency range which is lower than the 12–21% typically seen by silicon-based PVs. Because organic photovoltaics require very high purity and are relatively reactive they must be encapsulated which vastly increases the cost of manufacturing and means that they are not feasible for large scale-up. Dye-sensitized PVs are similar in efficiency to OPVs but are significantly easier to manufacture. However, these dye-sensitized photovoltaics present storage problems because the liquid electrolyte is toxic and can potentially permeate the plastics used in the cell. Quantum dot solar cells are solution-processed, meaning they are potentially scalable, but currently they peak at 12% efficiency.[117]
Organic and polymer photovoltaic (OPV) are a relatively new area of research. The tradition OPV cell structure layers consist of a semi-transparent electrode, electron blocking layer, tunnel junction, holes blocking layer, electrode, with the sun hitting the transparent electrode. OPV replaces silver with carbon as an electrode material lowering manufacturing cost and making them more environmentally friendly.[134] OPV are flexible, low weight, and work well with roll-to roll manufacturing for mass production.[135] OPV uses "only abundant elements coupled to an extremely low embodied energy through very low processing temperatures using only ambient processing conditions on simple printing equipment enabling energy pay-back times".[136] Current efficiencies range 1–6.5%,[57][137] however theoretical analyses show promise beyond 10% efficiency.[136]
Many different configurations of OPV exist using different materials for each layer. OPV technology rivals existing PV technologies in terms of EPBT even if they currently present a shorter operational lifetime. A 2013 study analyzed 12 different configurations all with 2% efficiency, the EPBT ranged from 0.29 to 0.52 years for 1 m2 of PV.[138] The average CO2-eq/kWh for OPV is 54.922 grams.[139]
Thermophotovoltaics
[edit]Thermophotovoltaic (TPV) energy conversion is a direct conversion process from heat to electricity via photons. A basic thermophotovoltaic system consists of a hot object emitting thermal radiation and a photovoltaic cell similar to a solar cell but tuned to the spectrum being emitted from the hot object.[140]
As TPV systems generally work at lower temperatures than solar cells, their efficiencies tend to be low. Offsetting this through the use of multi-junction cells based on non-silicon materials is common, but generally very expensive. This currently limits TPV to niche roles like spacecraft power and waste heat collection from larger systems like steam turbines.Solar module alignment
[edit]A number of solar modules may also be mounted vertically above each other in a tower, if the zenith distance of the Sun is greater than zero, and the tower can be turned horizontally as a whole and each module additionally around a horizontal axis. In such a tower the modules can follow the Sun exactly. Such a device may be described as a ladder mounted on a turnable disk. Each step of that ladder is the middle axis of a rectangular solar panel. In case the zenith distance of the Sun reaches zero, the "ladder" may be rotated to the north or the south to avoid a solar module producing a shadow on a lower one. Instead of an exactly vertical tower one can choose a tower with an axis directed to the polar star, meaning that it is parallel to the rotation axis of the Earth. In this case the angle between the axis and the Sun is always larger than 66 degrees. During a day it is only necessary to turn the panels around this axis to follow the Sun. Installations may be ground-mounted (and sometimes integrated with farming and grazing)[141] or built into the roof or walls of a building (building-integrated photovoltaics).
Where land may be limited, PV can be deployed as floating solar. In 2008 the Far Niente Winery pioneered the world's first "floatovoltaic" system by installing 994 photovoltaic solar panels onto 130 pontoons and floating them on the winery's irrigation pond.[142][143] A benefit of the set up is that the panels are kept at a lower temperature than they would be on land, leading to a higher efficiency of solar energy conversion. The floating panels also reduce the amount of water lost through evaporation and inhibit the growth of algae.[144]
Concentrator photovoltaics is a technology that contrary to conventional flat-plate PV systems uses lenses and curved mirrors to focus sunlight onto small, but highly efficient, multi-junction solar cells. These systems sometimes use solar trackers and a cooling system to increase their efficiency.
Efficiency
[edit]
In 2019, the world record for solar cell efficiency at 47.1% was achieved by using multi-junction concentrator solar cells, developed at National Renewable Energy Laboratory, Colorado, US.[145] The highest efficiencies achieved without concentration include a material by Sharp Corporation at 35.8% using a proprietary triple-junction manufacturing technology in 2009,[146] and Boeing Spectrolab (40.7% also using a triple-layer design).
There is an ongoing effort to increase the conversion efficiency of PV cells and modules, primarily for competitive advantage. In order to increase the efficiency of solar cells, it is important to choose a semiconductor material with an appropriate band gap that matches the solar spectrum. This will enhance the electrical and optical properties. Improving the method of charge collection is also useful for increasing the efficiency. There are several groups of materials that are being developed. Ultrahigh-efficiency devices (η>30%)[147] are made by using GaAs and GaInP2 semiconductors with multijunction tandem cells. High-quality, single-crystal silicon materials are used to achieve high-efficiency, low cost cells (η>20%).
Recent developments in organic photovoltaic cells (OPVs) have made significant advancements in power conversion efficiency from 3% to over 15% since their introduction in the 1980s.[148] To date, the highest reported power conversion efficiency ranges 6.7–8.94% for small molecule, 8.4–10.6% for polymer OPVs, and 7–21% for perovskite OPVs.[149][150] OPVs are expected to play a major role in the PV market. Recent improvements have increased the efficiency and lowered cost, while remaining environmentally-benign and renewable.
Several companies have begun embedding power optimizers into PV modules called smart modules. These modules perform maximum power point tracking (MPPT) for each module individually, measure performance data for monitoring, and provide additional safety features. Such modules can also compensate for shading effects, wherein a shadow falling across a section of a module causes the electrical output of one or more strings of cells in the module to decrease.[151]
One of the major causes for the decreased performance of cells is overheating. The efficiency of a solar cell declines by about 0.5% for every 1 degree Celsius increase in temperature. This means that a 100 degree increase in surface temperature could decrease the efficiency of a solar cell by about half. Self-cooling solar cells are one solution to this problem. Rather than using energy to cool the surface, pyramid and cone shapes can be formed from silica, and attached to the surface of a solar panel. Doing so allows visible light to reach the solar cells, but reflects infrared rays (which carry heat).[152]
Advantages
[edit]- Pollution and energy in production
The 122 PW of sunlight reaching the Earth's surface is plentiful—almost 10,000 times more than the 13 TW equivalent of average power consumed in 2005 by humans.[153] This abundance leads to the suggestion that it will not be long before solar energy will become the world's primary energy source.[154] Additionally, solar radiation has the highest power density (global mean of 170 W/m2) among renewable energies.[153][citation needed]
Solar power is pollution-free during use, which enables it to cut down on pollution when it is substituted for other energy sources. For example, MIT estimated that 52,000 people per year die prematurely in the U.S. from coal-fired power plant pollution[155] and all but one of these deaths could be prevented from using PV to replace coal.[156][157] Production end-wastes and emissions are manageable using existing pollution controls. End-of-use recycling technologies are under development[158] and policies are being produced that encourage recycling from producers.[159]
Solar panels are usually guaranteed for 25 years (but inverters tend to fail sooner),[160][161] with little maintenance or intervention after their initial set-up, so after the initial capital cost of building any solar power plant, operating costs are extremely low compared to existing power technologies.
Rooftop solar can be used locally, thus reducing transmission/distribution losses.[162]
- Solar cell research investment
Compared to fossil and nuclear energy sources, very little research money has been invested in the development of solar cells, so there is considerable room for improvement. Nevertheless, experimental high efficiency solar cells already have efficiencies of over 40% in case of concentrating photovoltaic cells[163] and efficiencies are rapidly rising while mass-production costs are rapidly falling.[164]
- Housing subsidies
In some states of the United States, much of the investment in a home-mounted system may be lost if the homeowner moves and the buyer puts less value on the system than the seller. The city of Berkeley developed an innovative financing method to remove this limitation, by adding a tax assessment that is transferred with the home to pay for the solar panels.[165] Now known as PACE, Property Assessed Clean Energy, 30 U.S. states have duplicated this solution.[166]
Disadvantages
[edit]- Impact on electricity network

For behind-the-meter rooftop photovoltaic systems, the energy flow becomes two-way. When there is more local generation than consumption, electricity is exported to the grid, allowing for net metering. However, electricity networks traditionally are not designed to deal with two-way energy transfer, which may introduce technical issues. An over-voltage issue may come out as the electricity flows from these PV households back to the network.[167] There are solutions to manage the over-voltage issue, such as regulating PV inverter power factor, new voltage and energy control equipment at electricity distributor level, re-conductor the electricity wires, demand side management, etc. There are often limitations and costs related to these solutions.
High generation during the middle of the day reduces the net generation demand, but higher peak net demand as the sun goes down can require rapid ramping of utility generating stations, producing a load profile called the duck curve.
See also
[edit]![]() | This section may contain an excessive amount of intricate detail that may interest only a particular audience. (September 2022) |
- Agrivoltaic
- American Solar Energy Society
- Anomalous photovoltaic effect
- Copper in renewable energy § Solar photovoltaic power generation
- Cost of electricity by source
- Energy demand management
- Electromotive force § Solar cell
- List of photovoltaics companies
- Photoelectrochemical cell
- Quantum efficiency § Quantum efficiency of solar cells
- Renewable energy commercialization
- Solar cell fabric
- Solar module quality assurance
- Solar photovoltaic monitoring
- Solar power by country
- Solar thermal energy
- Theory of solar cell
References
[edit]- ^ a b Lo Piano, Samuele; Mayumi, Kozo (2017). "Toward an integrated assessment of the performance of photovoltaic systems for electricity generation". Applied Energy. 186 (2): 167–74. doi:10.1016/j.apenergy.2016.05.102. S2CID 156783885.
- ^ "Solar Panels Reduce CO2 Emissions More Per Acre Than Trees — and Much More Than Corn Ethanol – State of the Planet". 26 October 2022. Retrieved 22 May 2024.
- ^ a b c d e f g h Bazilian, M.; Onyeji, I.; Liebreich, M.; MacGill, I.; Chase, J.; Shah, J.; Gielen, D.; Arent, D.; Landfear, D.; Zhengrong, S. (2013). "Re-considering the economics of photovoltaic power" (PDF). Renewable Energy. 53: 329–338. Bibcode:2013REne...53..329B. CiteSeerX 10.1.1.692.1880. doi:10.1016/j.renene.2012.11.029. Archived from the original (PDF) on 31 May 2014. Retrieved 4 September 2015.
- ^ Palz, Wolfgang (2013). Solar Power for the World: What You Wanted to Know about Photovoltaics. CRC Press. pp. 131–. ISBN 978-981-4411-87-5.
- ^ a b c d Roser, Max (1 December 2020). "Why did renewables become so cheap so fast?". Our World in Data.
- ^ Shubbak, Mahmood H. (2019). "The technological system of production and innovation: The case of photovoltaic technology in China". Research Policy. 48 (4): 993–1015. doi:10.1016/j.respol.2018.10.003. S2CID 158742469.
- ^ Renewable Energy Policy Network for the 21st century (REN21), Renewables 2010 Global Status Report Archived 13 September 2014 at the Wayback Machine, Paris, 2010, pp. 1–80.
- ^ "PHOTOVOLTAICS REPORT" (PDF). Fraunhofer Institute for Solar Energy Systems. 16 September 2020. p. 4.
- ^ "Renewables 2019". IEA. 21 October 2019. Retrieved 26 January 2020.
- ^ "KAHRAMAA and Siraj Energy Sign Agreements for Al-Kharsaah Solar PV Power Plant". Qatar General Electricity & Water Corporation "KAHRAMAA". 20 January 2020. Retrieved 26 January 2020.
- ^ Sunil Prasad Lohani, Andrew Blakers: 100% renewable energy with pumped-hydro-energy storage in Nepal. In: Clean Energy 5, 2, 2021, 243–253, doi:10.1093/ce/zkab011.
- ^ Smee, Alfred (1849). Elements of electro-biology,: or the voltaic mechanism of man; of electro-pathology, especially of the nervous system; and of electro-therapeutics. London: Longman, Brown, Green, and Longmans. p. 15.
- ^ Palz, Wolfgang (21 October 2013). Solar Power for the World: What You Wanted to Know about Photovoltaics. CRC Press. ISBN 978-981-4411-87-5.
- ^ Noguchi, Masa. "Number of Residential PV Installation in Japan: 1994-2003".
- ^ Photovoltaic Effect Archived 14 July 2011 at the Wayback Machine. Mrsolar.com. Retrieved 12 December 2010
- ^ The photovoltaic effect Archived 12 October 2010 at the Wayback Machine. Encyclobeamia.solarbotics.net. Retrieved on 12 December 2010.
- ^ Jacobson, Mark Z. (2009). "Review of Solutions to Global Warming, Air Pollution, and Energy Security". Energy & Environmental Science. 2 (2): 148–173. Bibcode:2009GeCAS..73R.581J. CiteSeerX 10.1.1.180.4676. doi:10.1039/B809990C.
- ^ German PV market. Solarbuzz.com. Retrieved on 3 June 2012.
- ^ BP Solar to Expand Its Solar Cell Plants in Spain and India[usurped]. Renewableenergyaccess.com. 23 March 2007. Retrieved on 3 June 2012.
- ^ Bullis, Kevin (23 June 2006). Large-Scale, Cheap Solar Electricity. Technologyreview.com. Retrieved on 3 June 2012.
- ^ Luque, Antonio & Hegedus, Steven (2003). Handbook of Photovoltaic Science and Engineering. John Wiley and Sons. ISBN 978-0-471-49196-5.
- ^ The PVWatts Solar Calculator Retrieved on 7 September 2012
- ^ Massachusetts: a Good Solar Market Archived 12 September 2012 at the Wayback Machine. Remenergyco.com. Retrieved on 31 May 2013.
- ^ a b Kannan, Nadarajah; Vakeesan, Divagar (1 September 2016). "Solar energy for future world: - A review". Renewable and Sustainable Energy Reviews. 62: 1092–1105. Bibcode:2016RSERv..62.1092K. doi:10.1016/j.rser.2016.05.022. ISSN 1364-0321.
- ^ Köberle, Alexandre C.; Gernaat, David E. H. J.; van Vuuren, Detlef P. (1 September 2015). "Assessing current and future techno-economic potential of concentrated solar power and photovoltaic electricity generation". Energy. 89: 739–756. Bibcode:2015Ene....89..739K. doi:10.1016/j.energy.2015.05.145. hdl:1874/319865. ISSN 0360-5442. S2CID 108996432.
- ^ a b Billy Roberts (20 October 2008). "Photovoltaic Solar Resource of the United States". National Renewable Energy Laboratory. Retrieved 17 April 2017.
- ^ David J. C. MacKay. "Sustainable Energy - without the hot air". inference.org.uk. Retrieved 20 November 2017.
Solar photovoltaics: data from a 25-m2 array in Cambridgeshire in 2006
- ^ a b Kumar, Ankush (3 January 2017). "Predicting efficiency of solar cells based on transparent conducting electrodes". Journal of Applied Physics. 121 (1): 014502. Bibcode:2017JAP...121a4502K. doi:10.1063/1.4973117. ISSN 0021-8979.
- ^ "Photovoltaic Cell Conversion Efficiency Basics". U.S. Department of Energy. Retrieved 6 September 2014.
- ^ Schygulla, Patrick; Beutel, Paul; Heckelmann, Stefan; Höhn, Oliver; Klitzke, Malte; Schön, Jonas; Oliva, Eduard; Predan, Felix; Schachtner, Michael; Siefer, Gerald; Helmers, Henning; Dimroth, Frank; Lackner, David (2022). Quadruple Junction Solar Cell with 47.6 % Conversion Efficiency under Concentration. International Conference on Metal Organic Vapor Phase Epitaxy 2022.
- ^ Helmers, Henning; Höhn, Oliver; Lackner, David; Schygulla, Patrick; Klitzke, Malte; Schön, Jonas; Pellegrino, Carmine; Oliva, Eduard; Schachtner, Michael; Beutel, Paul; Heckelmann, Stefan; Predan, Felix; Ohlmann, Jens; Siefer, Gerald; Dimroth, Frank (8 March 2024). Freundlich, Alexandre; Hinzer, Karin; Collin, Stéphane; Sellers, Ian R. (eds.). "Advancing solar energy conversion efficiency to 47.6% and exploring the spectral versatility of III-V photonic power converters". Proceedings Volume 12881, Physics, Simulation, and Photonic Engineering of Photovoltaic Devices XIII. 12881: 1288103. Bibcode:2024SPIE12881E..03H. doi:10.1117/12.3000352. ISBN 978-1-5106-7022-8.
- ^ Geisz, John F.; France, Ryan M.; Schulte, Kevin L.; Steiner, Myles A.; Norman, Andrew G.; Guthrey, Harvey L.; Young, Matthew R.; Song, Tao; Moriarty, Thomas (April 2020). "Six-junction III–V solar cells with 47.1% conversion efficiency under 143 Suns concentration". Nature Energy. 5 (4): 326–335. Bibcode:2020NatEn...5..326G. doi:10.1038/s41560-020-0598-5. ISSN 2058-7546. OSTI 1659948. S2CID 216289881.
- ^ Ozdemir, Derya (20 May 2022). "Scientists just broke the record for the highest efficiency solar cell". interestingengineering.com. Retrieved 7 August 2023.
- ^ France, Ryan M.; Geisz, John F.; Song, Tao; Olavarria, Waldo; Young, Michelle; Kibbler, Alan; Steiner, Myles A. (18 May 2022). "Triple-junction solar cells with 39.5% terrestrial and 34.2% space efficiency enabled by thick quantum well superlattices". Joule. 6 (5): 1121–1135. arXiv:2203.15593. Bibcode:2022Joule...6.1121F. doi:10.1016/j.joule.2022.04.024. ISSN 2542-4351. S2CID 247778421.
- ^ Dunlop, James P. (2012). Photovoltaic systems. National Joint Apprenticeship and Training Committee for the Electrical Industry (3rd ed.). Orland Park, IL: American Technical Publishers, Inc. ISBN 978-1-935941-05-7. OCLC 828685287.
- ^ Bowden, Stuart; Honsberg, Christiana. "Bypass Diodes". Photovoltaic Education. Retrieved 29 June 2021.
- ^ "Open-Circuit Voltage (Battery)". Electrical School. 13 June 2018. Retrieved 30 June 2021.
- ^ "REC Alpha Black Series Factsheet" (PDF).
- ^ "TSM PC/PM14 Datasheet" (PDF). Archived from the original (PDF) on 29 October 2013. Retrieved 4 June 2012.
- ^ "LBS Poly 260 275 Data sheet" (PDF). Archived from the original (PDF) on 9 January 2019. Retrieved 9 January 2018.
- ^ Piliougine, M.; Oukaja, A.; Sidrach-de-Cardona, M.; Spagnuolo, G. (2021). Temperature coefficients of degraded crystalline silicon photovoltaic modules at outdoor conditions. Vol. 29. pp. 558–570. doi:10.1002/pip.3396. S2CID 233976803.
{{cite book}}
:|work=
ignored (help) - ^ "Are Solar Panels Affected by Weather? - Energy Informative". Energy Informative. Retrieved 14 March 2018.
- ^ "Solarplaza Potential Induced Degradation: Combatting a Phantom Menace". www.solarplaza.com. Retrieved 4 September 2017.
- ^ (www.inspire.cz), INSPIRE CZ s.r.o. "What is PID? — eicero". eicero.com. Archived from the original on 4 September 2017. Retrieved 4 September 2017.
- ^ "How Solar Cells Work". HowStuffWorks. April 2000. Retrieved 9 December 2015.
- ^ "Bonding in Metals and Semiconductors". 2012books.lardbucket.org. Retrieved 9 December 2015.
- ^ Piliougine, M.; Oukaja, A.; Sánchez-Friera, P.; Petrone, G.; Sánchez-Pacheco, J.F.; Spagnuolo, G.; Sidrach-de-Cardona, M. (2021). "Analysis of the degradation of single-crystalline silicon modules after 21 years of operation". Progress in Photovoltaics: Research and Applications. Progress in Photovoltaics. 29 (8): 907–919. doi:10.1002/pip.3409. hdl:10630/29057. S2CID 234831264.
- ^ Piliougine, M.; Oukaja, A.; Sidrach-de-Cardona, M.; Spagnuolo, G. (2022). "Analysis of the degradation of amorphous silicon-based modules after 11 years of exposure by means of IEC60891:2021 procedure 3". Progress in Photovoltaics: Research and Applications. Progress in Photovoltaics. 30 (10): 1176–1187. doi:10.1002/pip.3567. hdl:10630/24064. S2CID 248487635.
- ^ Piliougine, M.; Sánchez-Friera, P.; Petrone, G.; Sánchez-Pacheco, J.F.; Spagnuolo, G.; Sidrach-de-Cardona, M. (2022). "New model to study the outdoor degradation of thin-film photovoltaic modules". Renewable Energy. 193: 857–869. Bibcode:2022REne..193..857P. doi:10.1016/j.renene.2022.05.063. hdl:10630/29061. S2CID 248926054.
- ^ Platzer, Michael (27 January 2015). "U.S. Solar Photovoltaic Manufacturing: Industry Trends, Global Competition, Federal Support". Congressional Research Service.
- ^ "How PV Cells Are Made". www.fsec.ucf.edu. Retrieved 5 November 2015.
- ^ "Are we headed for a solar waste crisis?". Environmentalprogress.org. 21 June 2017. Retrieved 30 December 2017.
- ^ a b Fthenakis, V. M., Kim, H. C. & Alsema, E. (2008). "Emissions from photovoltaic life cycles". Environmental Science & Technology. 42 (6): 2168–2174. Bibcode:2008EnST...42.2168F. doi:10.1021/es071763q. hdl:1874/32964. PMID 18409654. S2CID 20850468.
{{cite journal}}
: CS1 maint: multiple names: authors list (link) - ^ a b c d e f Collier, J., Wu, S. & Apul, D. (2014). "Life cycle environmental impacts from CZTS (copper zinc tin sulfide) and Zn3P2 (zinc phosphide) thin film PV (photovoltaic) cells". Energy. 74: 314–321. Bibcode:2014Ene....74..314C. doi:10.1016/j.energy.2014.06.076.
{{cite journal}}
: CS1 maint: multiple names: authors list (link) - ^ a b c d "An analysis of the energy efficiency of photovoltaic cells in reducing CO2 emissions" (PDF). clca.columbia.edu. 31 May 2009. Archived (PDF) from the original on 25 March 2015.
- ^ a b c d "PHOTOVOLTAICS REPORT" (PDF). Fraunhofer Institute for Solar Energy Systems. 16 September 2020. pp. 36, 43, 46.
- ^ a b Anctil, A., Babbitt, C. W., Raffaelle, R. P. & Landi, B. J. (2013). "Cumulative energy demand for small molecule and polymer photovoltaics". Progress in Photovoltaics: Research and Applications. 21 (7): 1541–1554. doi:10.1002/pip.2226. S2CID 94279905.
{{cite journal}}
: CS1 maint: multiple names: authors list (link) - ^ Ibon Galarraga, M. González-Eguino, Anil Markandya (1 January 2011). Handbook of Sustainable Energy. Edward Elgar Publishing. p. 37. ISBN 978-0-85793-638-7. Retrieved 9 May 2017 – via Google Books.
{{cite book}}
: CS1 maint: multiple names: authors list (link) - ^ a b Bhandari, K. P., Collier, J. M., Ellingson, R. J. & Apul, D. S. (2015). "Energy payback time (EPBT) and energy return on energy invested (EROI) of solar photovoltaic systems: A systematic review and meta-analysis". Renewable and Sustainable Energy Reviews. 47: 133–141. Bibcode:2015RSERv..47..133B. doi:10.1016/j.rser.2015.02.057.
{{cite journal}}
: CS1 maint: multiple names: authors list (link) - ^ Marco Raugei; Pere Fullana-i-Palmer; Vasilis Fthenakis (March 2012). "The Energy Return on Energy Investment (EROI) of Photovoltaics: Methodology and Comparisons with Fossil Fuel Life Cycles" (PDF). www.bnl.gov/. Archived (PDF) from the original on 8 March 2016.
- ^ Vasilis Fthenakis; Rolf Frischknecht; Marco Raugei; Hyung Chul Kim; Erik Alsema; Michael Held; Mariska de Wild-Scholten (November 2011). "Methodology Guidelines on Life Cycle Assessment of Photovoltaic Electricity" (PDF). www.iea-pvps.org/. IEA-PVPS. pp. 8–10. Archived (PDF) from the original on 24 September 2015.
- ^ "Production Process of Silicon". www.simcoa.com.au. Simcoa Operations. Archived from the original on 19 June 2014. Retrieved 17 September 2014.
- ^ "Reaching kerf loss below 100 μm by optimizations" (PDF). Fraunhofer ISE, 24th European PV Solar Energy Conference and Exhibition. September 2009.
- ^ "Silicon kerf loss recycling". HZDR - Helmholtz-Zentrum Dresden-Rossendorf. 4 April 2014.
- ^ "Life Cycle Assessment of Future Photovoltaic Electricity Production from Residential-scale Systems Operated in Europe". IEA-PVPS. 13 March 2015.
- ^ a b Life Cycle Greenhouse Gas Emissions from Solar Photovoltaics, National Renewable Energy Laboratory, U.S. Department of Energy, 2012, 1–2.
- ^ a b Krebs, F. C. (2009). "Fabrication and processing of polymer solar cells: a review of printing and coating techniques". Solar Energy Materials and Solar Cells. 93 (4): 394–412. Bibcode:2009SEMSC..93..394K. doi:10.1016/j.solmat.2008.10.004.
- ^ a b Yue D.; You F.; Darling S. B. (2014). "Domestic and overseas manufacturing scenarios of silicon-based photovoltaics: Life cycle energy and environmental comparative analysis". Solar Energy. 105: 669–678. Bibcode:2014SoEn..105..669Y. doi:10.1016/j.solener.2014.04.008.
- ^ Gaidajis, G. & Angelakoglou, K. (2012). "Environmental performance of renewable energy systems with the application of life-cycle assessment: a multi-Si photovoltaic module case study". Civil Engineering and Environmental Systems. 29 (4): 231–238. Bibcode:2012CEES...29..231G. doi:10.1080/10286608.2012.710608. S2CID 110058349.
- ^ Photovoltaics Report. (Fraunhofer Institute for Solar Energy Systems, ISE, 2015).
- ^ a b Goe, M. & Gaustad, G. (2014). "Strengthening the case for recycling photovoltaics: An energy payback analysis". Applied Energy. 120: 41–48. Bibcode:2014ApEn..120...41G. doi:10.1016/j.apenergy.2014.01.036.
- ^ Brown, G. F. & Wu, J. (2009). "Third generation photovoltaics". Laser & Photonics Reviews. 3 (4): 394–405. Bibcode:2009LPRv....3..394B. doi:10.1002/lpor.200810039. S2CID 13179665.
- ^ a b Celik, I., Mason, B. E., Phillips, A. B., Heben, M. J., & Apul, D. S. (2017). Environmental Impacts from Photovoltaic Solar Cells Made with Single Walled Carbon Nanotubes. Environmental Science & Technology.
- ^ Agboola, A. E. Development and model formulation of scalable carbon nanotube processes: HiPCO and CoMoCAT process models;Louisiana State University, 2005.
- ^ Wadia, C., Alivisatos, A. P. & Kammen, D. M. (2009). "Materials Availability Expands the Opportunity for Large-Scale Photovoltaics Deployment". Environmental Science and Technology. 43 (6): 2072–2077. Bibcode:2009EnST...43.2072W. doi:10.1021/es8019534. PMID 19368216.
{{cite journal}}
: CS1 maint: multiple names: authors list (link) - ^ Alharbi, Fahhad; Bass, John D.; Salhi, Abdelmajid; Alyamani, Ahmed; Kim, Ho-Cheol; Miller, Robert D. (2011). "Abundant non-toxic materials for thin film solar cells: Alternative to conventional materials". Renewable Energy. 36 (10): 2753–2758. Bibcode:2011REne...36.2753A. doi:10.1016/j.renene.2011.03.010.
- ^ "Insolation Levels (Europe)". Apricus Solar. Archived from the original on 17 April 2012. Retrieved 14 April 2012.
- ^ Renewable energy costs drop in '09 Reuters, 23 November 2009.
- ^ Solar Power 50% Cheaper By Year End – Analysis. Reuters, 24 November 2009.
- ^ a b Harris, Arno (31 August 2011). "A Silver Lining in Declining Solar Prices". Renewable Energy World.
- ^ Renewable Power Generation Costs in 2019. Abu Dhabi: International Renewable Energy Agency. 2020. ISBN 978-92-9260-244-4.
- ^ L. Liu, W. Miller, and G. Ledwich. (2017) Solutions for reducing facilities electricity costs. Australian Ageing Agenda. 39-40. Available: https://www.australianageingagenda.com.au/2017/10/27/solutions-reducing-facility-electricity-costs/ Archived 20 May 2019 at the Wayback Machine
- ^ Miller, Wendy; Liu, Lei Aaron; Amin, Zakaria; Gray, Matthew (2018). "Involving occupants in net-zero-energy solar housing retrofits: An Australian sub-tropical case study". Solar Energy. 159: 390–404. Bibcode:2018SoEn..159..390M. doi:10.1016/j.solener.2017.10.008.
- ^ "Solar (photovoltaic) panel prices vs. cumulative capacity". OurWorldInData.org. 2024. Archived from the original on 24 January 2025. OWID credits source data to: Nemet (2009); Farmer & Lafond (2016); International Renewable Energy Agency (IRENA, 2024).
- ^ a b c "Sunny Uplands: Alternative energy will no longer be alternative". The Economist. 21 November 2012. Retrieved 28 December 2012.
- ^ a b Quiggin, John (3 January 2012). "The End of the Nuclear Renaissance". National Interest.
- ^ a b Wells, Ken (25 October 2012). "Solar Energy Is Ready. The U.S. Isn't". Bloomberg Businessweek. Archived from the original on 27 October 2012. Retrieved 1 November 2012.
- ^ Solar PV Module Costs to Fall to 36 Cents per Watt by 2017. Greentechmedia.com (2013-06-18). Retrieved on 2015-04-15.
- ^ a b Alafita, T.; Pearce, J. M. (2014). "Securitization of residential solar photovoltaic assets: Costs, risks and uncertainty". Energy Policy. 67: 488–498. Bibcode:2014EnPol..67..488A. doi:10.1016/j.enpol.2013.12.045. S2CID 11079398.
- ^ Liebreich, Michael (29 January 2014). "A YEAR OF CRACKING ICE: 10 PREDICTIONS FOR 2014". Bloomberg New Energy Finance. Retrieved 24 April 2014.
- ^ "2014 Outlook: Let the Second Gold Rush Begin" (PDF). Deutsche Bank Markets Research. 6 January 2014. Archived (PDF) from the original on 29 November 2014. Retrieved 22 November 2014.
- ^ Crooks, Ed (5 April 2024). "China's solar growth sends module prices plummeting". Wood Mackenzie. Retrieved 12 July 2024.
- ^ a b c d Branker, K.; Pathak, M.J.M.; Pearce, J.M. (2011). "A Review of Solar Photovoltaic Levelized Cost of Electricity". Renewable and Sustainable Energy Reviews. 15 (9): 4470–4482. Bibcode:2011RSERv..15.4470B. doi:10.1016/j.rser.2011.07.104. hdl:1974/6879. S2CID 73523633.
- ^ "Renewables Investment Breaks Records". Renewable Energy World. 29 August 2011.
- ^ Hockenos, Paul (10 February 2021). "Is Germany Making Too Much Renewable Energy?". Foreign Policy. Retrieved 7 March 2021.
- ^ a b Nancy M. Haegel (2017). "Terawatt-scale photovoltaics: Trajectories and challenges". Science. 356 (6334): 141–143. Bibcode:2017Sci...356..141H. doi:10.1126/science.aal1288. hdl:10945/57762. OSTI 1352502. PMID 28408563. S2CID 206654326.
- ^ Adeh, Elnaz H.; Good, Stephen P.; Calaf, M.; Higgins, Chad W. (7 August 2019). "Solar PV Power Potential is Greatest Over Croplands". Scientific Reports. 9 (1): 11442. Bibcode:2019NatSR...911442A. doi:10.1038/s41598-019-47803-3. ISSN 2045-2322. PMC 6685942. PMID 31391497.
- ^ TROMMSDORFF, Maximillian (2016). "An economic analysis of agrophotovoltaics: Opportunities, risks and strategies towards a more efficient land use" (PDF). The Constitutional Economics Network Working Papers.
- ^ "Cost of capital survey shows investments in solar PV can be less risky than gas power in emerging and developing economies, though values remain high – Analysis". IEA. 30 November 2023. Retrieved 10 December 2024.
- ^ Lowder, T., & Mendelsohn, M. (2013). The Potential of Securitization in Solar PV Finance.[page needed]
- ^ "Solar Securitization for Rwanda". The Global Innovation Lab for Climate Finance (in Brazilian Portuguese). Retrieved 10 December 2024.
- ^ Matar, Walid; Anwer, Murad (2017). "Jointly reforming the prices of industrial fuels and residential electricity in Saudi Arabia". Energy Policy. 109: 747–756. Bibcode:2017EnPol.109..747M. doi:10.1016/j.enpol.2017.07.060.
- ^ Utilities' Honest Assessment of Solar in the Electricity Supply. Greentechmedia.com (7 May 2012). Retrieved on 31 May 2013.
- ^ Straza; Schneegans (11 June 2021). Are we using science for smarter development?. Paris: UNESCO. ISBN 978-92-3-100450-6.
- ^ "Snapshot of Global Photovoltaic Markets 2017" (PDF). report. International Energy Agency. 19 April 2017. Retrieved 11 July 2017.
- ^ "Snapshot of Global PV 1992–2014" (PDF). International Energy Agency — Photovoltaic Power Systems Programme. 30 March 2015. Archived from the original on 7 April 2015.
- ^ "Renewables 2011: Global Status Report". REN21. 2011. p. 22. Archived from the original on 13 September 2014. Retrieved 31 May 2013.
- ^ "Snapshot 2020". IEA-PVPS. 20 April 2020.
- ^ a b c d Do, Thang Nam; Burke, Paul J.; Baldwin, Kenneth G. H.; Nguyen, Chinh The (1 September 2020). "Underlying drivers and barriers for solar photovoltaics diffusion: The case of Vietnam". Energy Policy. 144: 111561. Bibcode:2020EnPol.14411561D. doi:10.1016/j.enpol.2020.111561. hdl:1885/206307. ISSN 0301-4215. S2CID 225245522.
- ^ "China to add 55-65 GW of solar power capacity in 2021 -industry body". Reuters. 23 July 2021. Retrieved 15 October 2022.
- ^ a b "Snapshot 2020 – IEA-PVPS". iea-pvps.org. 20 April 2020. Retrieved 10 May 2020.
- ^ Solar Photovoltaic Electricity Empowering the World Archived 22 August 2012 at the Wayback Machine. Epia.org (22 September 2012). Retrieved on 31 May 2013.
- ^ Haugan, H. J.; Elhamri, S.; Szmulowicz, F.; Ullrich, B.; Brown, G. J.; Mitchel, W. C. (2008). "Study of residual background carriers in midinfrared InAs/GaSb superlattices for uncooled detector operation". Applied Physics Letters. 92 (7): 071102. Bibcode:2008ApPhL..92g1102H. doi:10.1063/1.2884264. S2CID 39187771.
- ^ "Solar PV Modules". www.targray.com. Retrieved 3 October 2018.
- ^ Kosasih, Felix Utama; Rakocevic, Lucija; Aernouts, Tom; Poortmans, Jef; Ducati, Caterina (11 December 2019). "Electron Microscopy Characterization of P3 Lines and Laser Scribing-Induced Perovskite Decomposition in Perovskite Solar Modules". ACS Applied Materials & Interfaces. 11 (49): 45646–45655. doi:10.1021/acsami.9b15520. PMID 31663326. S2CID 204967452.
- ^ Di Giacomo, Francesco; Castriotta, Luigi A.; Kosasih, Felix U.; Di Girolamo, Diego; Ducati, Caterina; Di Carlo, Aldo (20 December 2020). "Upscaling Inverted Perovskite Solar Cells: Optimization of Laser Scribing for Highly Efficient Mini-Modules". Micromachines. 11 (12): 1127. doi:10.3390/mi11121127. PMC 7767295. PMID 33419276.
- ^ a b Matteocci, Fabio; Vesce, Luigi; Kosasih, Felix Utama; Castriotta, Luigi Angelo; Cacovich, Stefania; Palma, Alessandro Lorenzo; Divitini, Giorgio; Ducati, Caterina; Di Carlo, Aldo (17 July 2019). "Fabrication and Morphological Characterization of High-Efficiency Blade-Coated Perovskite Solar Modules". ACS Applied Materials & Interfaces. 11 (28): 25195–25204. doi:10.1021/acsami.9b05730. PMID 31268662. S2CID 206497286.
- ^ "Thin Film Photovoltaics". www.fsec.ucf.edu. Retrieved 5 November 2015.
- ^ Best Research Cell Efficiences. nrel.gov (16 September 2019). Retrieved on 31 October 2019.
- ^ Eisenberg, D. A., Yu, M., Lam, C. W., Ogunseitan, O. A. & Schoenung, J. M. (2013). "Comparative alternative materials assessment to screen toxicity hazards in the life cycle of CIGS thin film photovoltaics". Journal of Hazardous Materials. 260: 534–542. Bibcode:2013JHzM..260..534E. doi:10.1016/j.jhazmat.2013.06.007. PMID 23811631. S2CID 26540719.
{{cite journal}}
: CS1 maint: multiple names: authors list (link) - ^ Kim, H. C., Fthenakis, V., Choi, J. K. & Turney, D. E. (2012). "Life cycle greenhouse gas emissions of thin-film photovoltaic electricity generation". Journal of Industrial Ecology. 16: S110 – S121. doi:10.1111/j.1530-9290.2011.00423.x. S2CID 153386434.
{{cite journal}}
: CS1 maint: multiple names: authors list (link) - ^ Werner, Jürgen H.; Zapf-Gottwick, R.; Koch, M.; Fischer, K. (2011). Toxic substances in photovoltaic modules. Proceedings of the 21st International Photovoltaic Science and Engineering Conference. Vol. 28. Fukuoka, Japan.
- ^ a b Manser, Joseph S.; Christians, Jeffrey A.; Kamat, Prashant V. (2016). "Intriguing Optoelectronic Properties of Metal Halide Perovskites". Chemical Reviews. 116 (21): 12956–13008. doi:10.1021/acs.chemrev.6b00136. PMID 27327168.
- ^ Hamers, Laurel (26 July 2017). "Perovskites power up the solar industry". Science News.
- ^ Kojima, Akihiro; Teshima, Kenjiro; Shirai, Yasuo; Miyasaka, Tsutomu (6 May 2009). "Organometal Halide Perovskites as Visible-Light Sensitizers for Photovoltaic Cells". Journal of the American Chemical Society. 131 (17): 6050–6051. doi:10.1021/ja809598r. PMID 19366264.
- ^ a b "Best Research-Cell Efficiencies" (PDF). National Renewable Energy Laboratory. 30 June 2022. Archived from the original (PDF) on 3 August 2022. Retrieved 12 July 2022.
- ^ Min, Hanul; Lee, Do Yoon; Kim, Junu; Kim, Gwisu; Lee, Kyoung Su; Kim, Jongbeom; Paik, Min Jae; Kim, Young Ki; Kim, Kwang S.; Kim, Min Gyu; Shin, Tae Joo; Il Seok, Sang (21 October 2021). "Perovskite solar cells with atomically coherent interlayers on SnO2 electrodes". Nature. 598 (7881): 444–450. Bibcode:2021Natur.598..444M. doi:10.1038/s41586-021-03964-8. PMID 34671136. S2CID 239052065.
- ^ Helmholtz-Zentrum Berlin für Materialien und Energie. "World record again at HZB: Almost 30 % efficiency for next-generation tandem solar cells". HZB Website.
- ^ Sun, Kai; Wang, Yanyan; Xu, Haoyuan; Zhang, Jing; Zhu, Yuejin; Hu, Ziyang (2019). "Short-Term Stability of Perovskite Solar Cells Affected by In Situ Interface Modification". Solar RRL. 3 (9): 1900089. doi:10.1002/solr.201900089. S2CID 202229877.
- ^ Goodson, Flynt (2014). "Supramolecular Multichromophoric Dye Sensitized Solar Cells". Archived from the original on 9 December 2022.
{{cite journal}}
: Cite journal requires|journal=
(help) - ^ Freitag, Marina; Teuscher, Joël; Saygili, Yasemin; Zhang, Xiaoyu; Giordano, Fabrizio; Liska, Paul; Hua, Jianli; Zakeeruddin, Shaik M.; Moser, Jacques-E.; Grätzel, Michael; Hagfeldt, Anders (17 June 2017). "Dye-sensitized solar cells for efficient power generation under ambient lighting". Nature Photonics. 11 (6): 372–378. Bibcode:2017NaPho..11..372F. doi:10.1038/nphoton.2017.60. S2CID 10780585 – via www.nature.com.
- ^ Raga, Sonia R.; Fabregat-Santiago, Francisco (23 January 2013). "Temperature effects in dye-sensitized solar cells". Physical Chemistry Chemical Physics. 15 (7): 2328–2336. Bibcode:2013PCCP...15.2328R. doi:10.1039/C2CP43220J. PMID 23295858 – via pubs.rsc.org.
- ^ Nikolaidou, Katerina; Sarang, Som; Ghosh, Sayantani (2019). "Nanostructured photovoltaics". Nano Futures. 3 (1): 012002. Bibcode:2019NanoF...3a2002N. doi:10.1088/2399-1984/ab02b5. S2CID 162176556.
- ^ Dos Reis Benatto, Gisele A.; Roth, Bérenger; Madsen, Morten V.; Hösel, Markus; Søndergaard, Roar R.; Jørgensen, Mikkel; Krebs, Frederik C. (2014). "Carbon: The Ultimate Electrode Choice for Widely Distributed Polymer Solar Cells". Advanced Energy Materials. 4 (15): n/a. Bibcode:2014AdEnM...400732D. doi:10.1002/aenm.201400732. S2CID 96990654.
- ^ Lattante, Sandro (2014). "Electron and Hole Transport Layers: Their Use in Inverted Bulk Heterojunction Polymer Solar Cells". Electronics. 3: 132–164. doi:10.3390/electronics3010132.
- ^ a b Krebs, Frederik C.; Jørgensen, Mikkel (2013). "Polymer and organic solar cells viewed as thin film technologies: What it will take for them to become a success outside academia". Solar Energy Materials and Solar Cells. 119: 73–76. Bibcode:2013SEMSC.119...73K. doi:10.1016/j.solmat.2013.05.032.
- ^ Espinosa, Nieves; García-Valverde, Rafael; Urbina, Antonio; Krebs, Frederik C. (2011). "A life cycle analysis of polymer solar cell modules prepared using roll-to-roll methods under ambient conditions". Solar Energy Materials and Solar Cells. 95 (5): 1293–1302. Bibcode:2011SEMSC..95.1293E. doi:10.1016/j.solmat.2010.08.020.
- ^ Espinosa, Nieves; Lenzmann, Frank O.; Ryley, Stephen; Angmo, Dechan; Hösel, Markus; Søndergaard, Roar R.; Huss, Dennis; Dafinger, Simone; Gritsch, Stefan; Kroon, Jan M.; Jørgensen, Mikkel; Krebs, Frederik C. (2013). "OPV for mobile applications: An evaluation of roll-to-roll processed indium and silver free polymer solar cells through analysis of life cycle, cost and layer quality using inline optical and functional inspection tools". Journal of Materials Chemistry A. 1 (24): 7037. doi:10.1039/C3TA01611K.
- ^ García-Valverde, R.; Miguel, C.; Martínez-Béjar, R.; Urbina, A. (2009). "Life cycle assessment study of a 4.2k Wp stand-alone photovoltaic system". Solar Energy. 83 (9): 1434–1445. Bibcode:2009SoEn...83.1434G. doi:10.1016/j.solener.2009.03.012.
- ^ Bauer, Thomas (2011). Thermophotovoltaics. Green Energy and Technology. doi:10.1007/978-3-642-19965-3. ISBN 978-3-642-19964-6.[page needed]
- ^ GE Invests, Delivers One of World's Largest Solar Power Plants. Huliq.com (12 April 2007). Retrieved on 3 June 2012.
- ^ Winery goes solar with 'Floatovoltaics'. SFGate (29 May 2008). Retrieved on 31 May 2013.
- ^ NAPA VALLEY'S FAR NIENTE WINERY INTRODUCES FIRST-EVER "FLOATOVOLTAIC" SOLAR ARRAY Archived 16 March 2015 at the Wayback Machine. farniente.com
- ^ Napa Winery Pioneers Solar Floatovoltaics. Forbes (18 April 2012). Retrieved on 31 May 2013.
- ^ Geisz, John F.; France, Ryan M.; Schulte, Kevin L.; Steiner, Myles A.; Norman, Andrew G.; Guthrey, Harvey L.; Young, Matthew R.; Song, Tao; Moriarty, Thomas (April 2020). "Six-junction III–V solar cells with 47.1% conversion efficiency under 143 Suns concentration". Nature Energy. 5 (4): 326–335. Bibcode:2020NatEn...5..326G. doi:10.1038/s41560-020-0598-5. ISSN 2058-7546. OSTI 1659948. S2CID 216289881.
- ^ Sharp Develops Solar Cell with World's Highest Conversion Efficiency of 35.8%. Physorg.com. 22 October 2009. Retrieved on 3 June 2012.
- ^ Deb, Satyen K. (May 2000) Recent Developments in High Efficiency PV cells. nrel.gov
- ^ Yu, J.; Zheng, Y.; Huang, J. (2014). "Towards High Performance Organic Photovoltaic Cells: A Review of Recent Development in Organic Photovoltaics". Polymers. 6 (9): 2473–2509. doi:10.3390/polym6092473.
- ^ Sun, Y.; Welch, G. C.; Leong, W. L.; Takacs, C. J.; Bazan, G. C.; Heeger, A. J. (2011). "Solution-processed small-molecule solar cells with 6.7% efficiency". Nature Materials. 11 (1): 44–8. Bibcode:2012NatMa..11...44S. doi:10.1038/nmat3160. PMID 22057387.
- ^ EPFL Achieves 21% Efficiency for Perovskites. dyesol.com (8 December 2015)
- ^ St. John, Jeff (23 August 2012) Solar Electronics, Panel Integration and the Bankability Challenge. greentechmedia.com
- ^ Self-cooling Solar Cells. CNN. 2014-09-18
- ^ a b Smil, Vaclav (2006) Energy at the Crossroads. oecd.org. Retrieved on 3 June 2012.
- ^ Renewable Energy: Is the Future in Nuclear? Archived 16 January 2014 at the Wayback Machine Prof. Gordon Aubrecht (Ohio State at Marion) TEDxColumbus, The Innovators – 18 October 2012
- ^ "Study: Air pollution causes 200,000 early deaths each year in the U.S". News.mit.edu. 29 August 2013. Retrieved 30 December 2017.
- ^ "The US could prevent a lot of deaths by switching from coal to solar". USA TODAY. Retrieved 30 December 2017.
- ^ Potential lives saved by replacing coal with solar photovoltaic electricity production in the U.S. Renewable and Sustainable Energy Reviews 80 (2017), pp. 710–715. open access
- ^ Nieuwlaar, Evert and Alsema, Erik. Environmental Aspects of PV Power Systems. IEA PVPS Task 1 Workshop, 25–27 June 1997, Utrecht, The Netherlands
- ^ McDonald, N.C.; Pearce, J.M. (2010). "Producer Responsibility and Recycling Solar Photovoltaic Modules" (PDF). Energy Policy. 38 (11): 7041–7047. Bibcode:2010EnPol..38.7041M. doi:10.1016/j.enpol.2010.07.023.
- ^ "How long do residential solar panels last?". pv magazine International. 23 July 2024. Retrieved 10 December 2024.
- ^ "US solar farms are aging. Is it time to begin repowering?". Utility Dive. Retrieved 10 December 2024.
- ^ U.S. Climate Change Technology Program – Transmission and Distribution Technologies Archived 27 September 2007 at the Wayback Machine. (PDF) . Retrieved on 3 June 2012.
- ^ Fraunhofer: 41.1% efficiency multi-junction solar cells. renewableenergyfocus.com (28 January 2009).
- ^ Study Sees Solar Cost-Competitive In Europe By 2015. Solar Cells Info (16 October 2007). Retrieved on 3 June 2012.
- ^ "Berkeley FIRST Solar Financing – City of Berkeley, CA". cityofberkeley.info. Archived from the original on 2 June 2013. Retrieved 9 February 2009.
- ^ DSIRE Solar Portal Archived 9 March 2012 at the Wayback Machine. Dsireusa.org (4 April 2011). Retrieved on 3 June 2012.
- ^ Miller, Wendy; Liu, Aaron; Amin, Zakaria; Wagner, Andreas (2018). "Power Quality and Rooftop-Photovoltaic Households: An Examination of Measured Data at Point of Customer Connection". Sustainability. 10 (4): 1224. Bibcode:2018Sust...10.1224M. doi:10.3390/su10041224.